Table of Contents (click to expand)
Asteroseismology, the study of stellar oscillations, deciphers the rhythmic vibrations of distant stars, shedding light on their inner dynamics and evolution. It offers a unique and invaluable window into the cosmos, enhancing our understanding of celestial bodies without the need for direct physical exploration.
Have you ever wondered how we can unravel the mysteries of distant stars without physically visiting them?
Imagine a scientific method that permits us to eavesdrop on the whispers of stars as they divulge their innermost secrets through the subtle language of vibrations.
What exactly are these rhythmic vibrations, and how do they contribute to our comprehension of the cosmos?
In this scientific inquiry, let’s learn more about Asteroseismology, the study of stellar oscillations, revealing their vital significance and the impact they have across various dimensions of astrophysics.
Recommended Video for you:
What Is Asteroseismology?
Asteroseismology offers a unique glimpse into star interiors and their evolution. Instead of solely relying on the light that stars emit, scientists decode the vibrations they generate.
These vibrations, known as stellar oscillations, are born from the interplay of gravity, pressure, and nuclear reactions within a star’s core.
The essence of asteroseismology revolves around dissecting these vibrations. Scientists bring valuable information about a star’s inner dynamics to light by examining the frequencies and patterns.
Through these vibrations, we can gain insights into temperature variations, density gradients, and the distribution of chemical elements within the star.
Decoding Stellar Oscillations
Imagine a bowl of water. If you gently touch the surface of the water, you will create a wave that travels outward across the surface. This wave is a sound wave, and is caused by the pressure variations in the water.
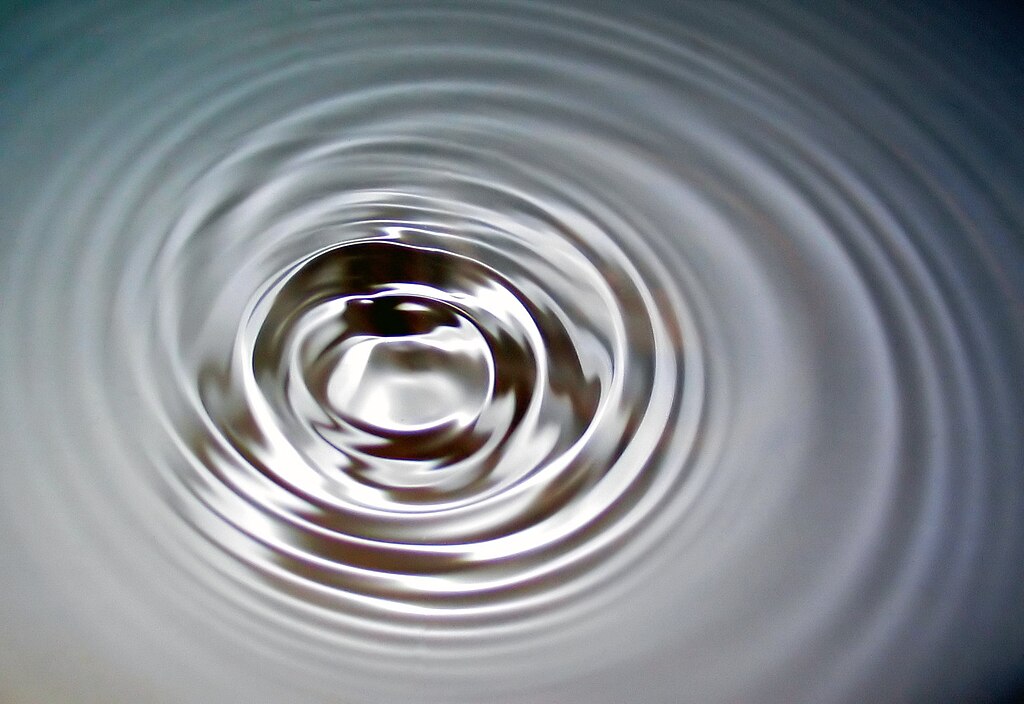
The same thing happens in stars.
Think of stars as gigantic balloons filled with hot, glowing gas. Deep inside the star, at its core, there’s an incredible amount of pressure. It’s akin to being at the bottom of a deep ocean where the water pressure is incredibly intense. This strong pressure at the center of the star is caused by the immense gravitational pull from all the star’s material.
Thus, the pressure inside a star is not constant. It is higher in the core and lower in the outer layers. This pressure gradient causes sound waves to travel through the star.
As we delve into the complex dynamics within a star, it’s important to introduce the concept of p-modes and g-modes, which play crucial roles in deciphering a star’s inner workings.
P-modes are like trapped sound waves inside the star. You can think of them like ripples in a water bowl, but they can’t escape, because the star’s gravity keeps them inside, as though there is a lid on the bowl.
Now, imagine that you added some oil on top of the water in the bowl. If you drop a stone into the bowl now, you’ll still create ripples in the water, but the oil layer will also move around like wavy jelly. This movement of the oil is the same as the g-mode in a star.
The buoyancy force in a star is like the force of gravity acting on the oil layer. The oil layer is denser than the water, so it tends to sink to the bottom of the pool. However, sound waves create disturbances in the oil layer, causing it to slosh around. The buoyancy force then acts to restore the oil layer to its equilibrium position.
In a similar way, the buoyancy force in a star acts to restore the equilibrium state of the star after a g-mode disturbance. The g-mode oscillation causes the hot, less dense material to rise to the surface of the star, while the cold, denser material sinks to the core. The buoyancy force then acts to reverse this process, causing the hot material to sink and the cold material to rise.
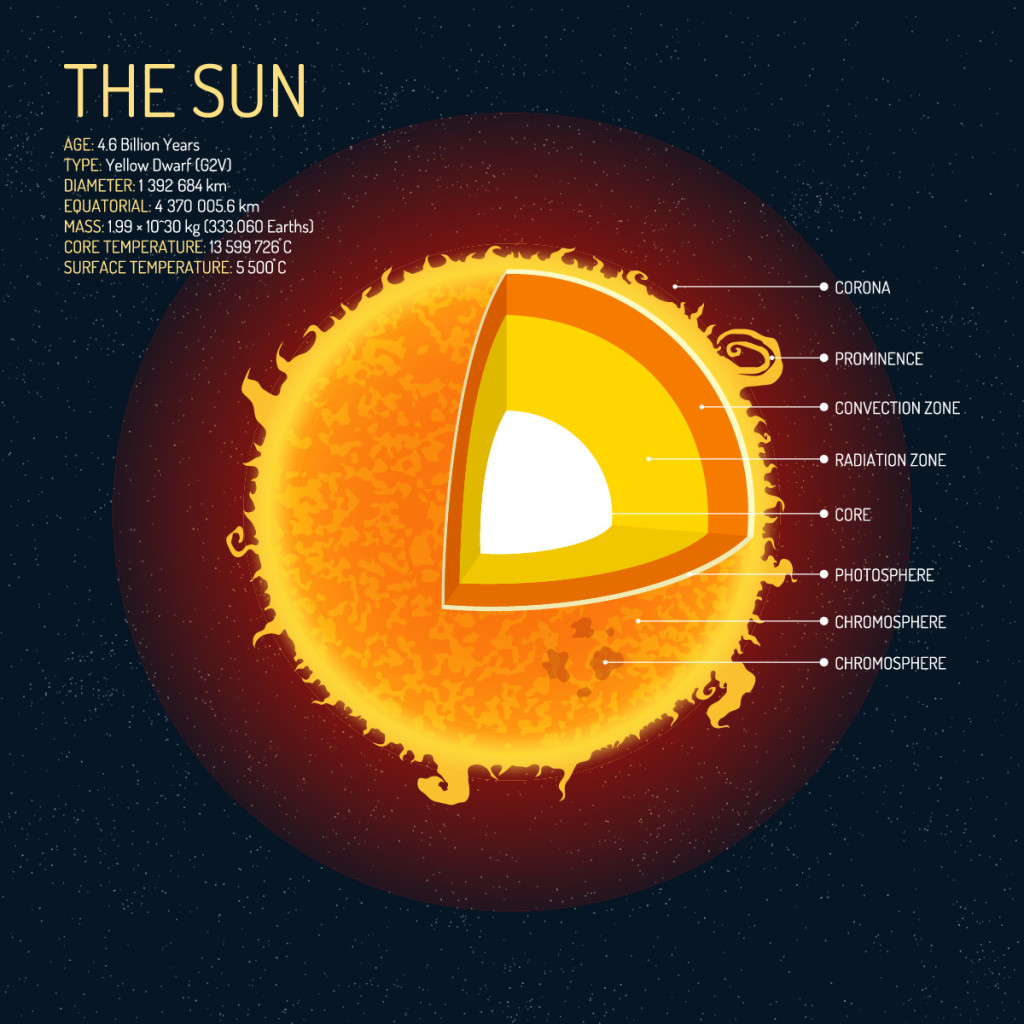
The p-modes can travel through the interior of a star, although opacity, stellar rotation, and the magnetic field of the stellar material can affect their propagation. The more opaque the material, the slower the p-modes travel. The stronger the magnetic field, the higher the frequency of the p-modes.
At the same time, pure g-modes are sound waves trapped deep inside a star’s radiative zone. The radiative zone is the region of a star where the heat is transported by radiation, rather than by convection. The g-modes emerge from the interplay between buoyancy and gravity. They penetrate deep into a star’s core, revealing its most intimate attributes.
The g-modes are evanescent in the outer convective zone of solar-like stars, meaning that they are quickly extinguished as they travel through the convective zone. They are less common than p-modes and are only observed in stars with deep convective zones.
Both p-modes and g-modes can be used to study the internal structure of stars. Astronomers can learn about a star’s density, temperature, and composition by measuring the frequencies and amplitudes of the oscillations.
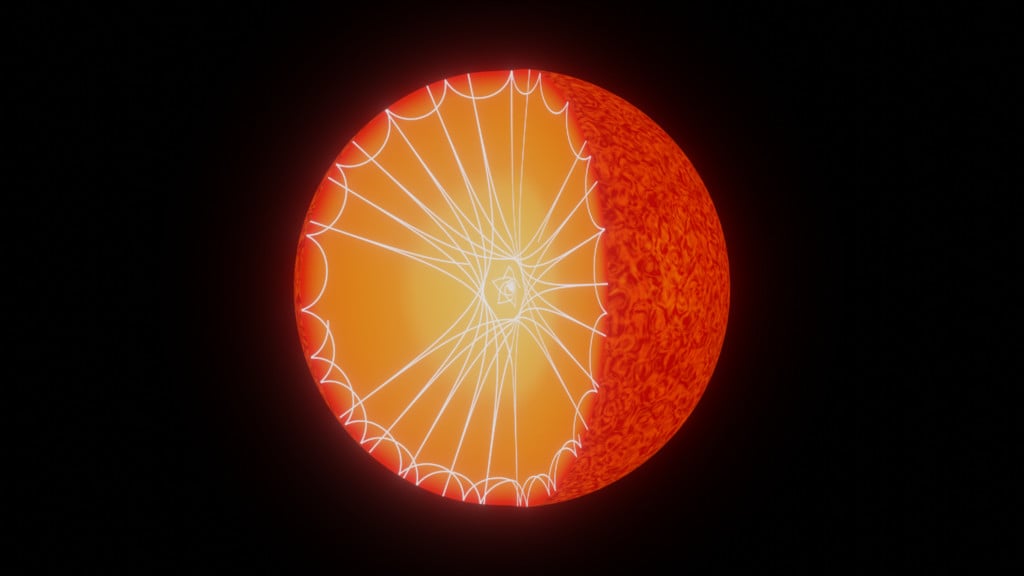
The interplay between p-modes and g-modes gives rise to mixed modes. These mixed modes occur when the physical separation between the cavities of p-modes and g-modes becomes minimal or overlaps. These cavities are where the modes are trapped.
The p-modes are more sensitive to the star’s outer layers, whereas g-modes are more sensitive to the star’s inner layers. Mixed modes, on the other hand, are sensitive to both the outer and inner layers of the star, making them very useful for studying the star’s profound internal structure.
Observational Insights
Now, without a medium, it’s obvious to those that understand the cosmos that the sound can’t travel, so how are we studying these vibrations? Well, the vibrations in the stars actually change their brightness, and that’s what the telescopes try to capture through different tools and metrics, one of which is the Doppler effect.
These variations due to the sound waves are extremely weak. Since the atmosphere can introduce noise into the observations, it is difficult for ground-based telescopes to detect these minute variations. However, some of their advantages include their capacity to be used to observe a wider range of stars, and the fact that they’re cheaper to build and operate.
One particularly famous ground-based telescope project is the Apache Point Observatory Galactic Evolution Experiment (APOGEE).
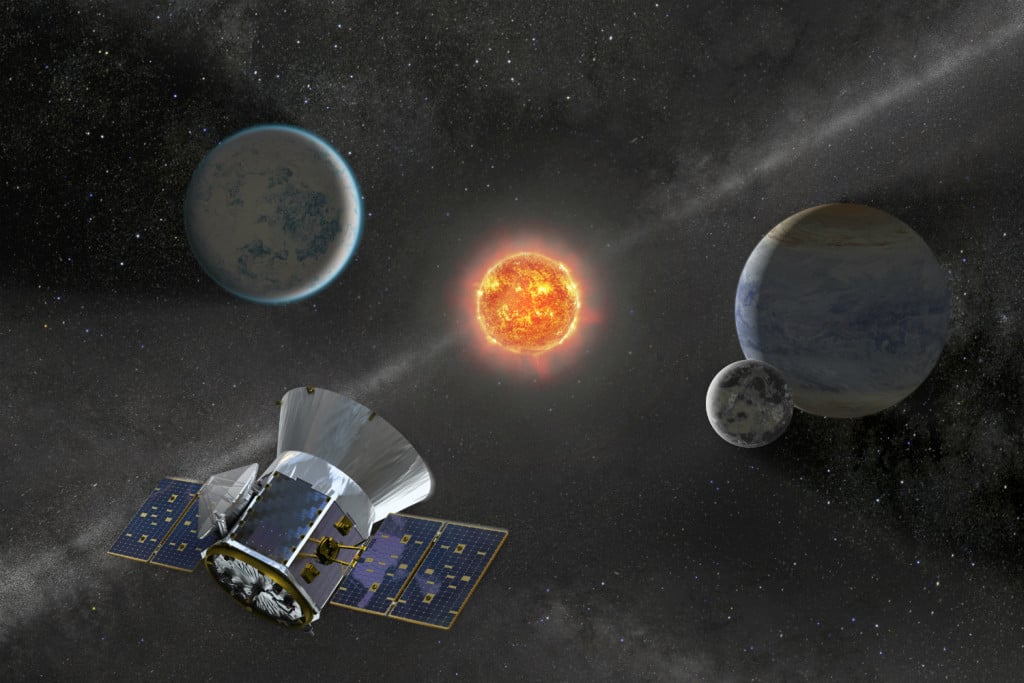
Astronomers tackle space-based telescopes, such as NASA’s Transiting Exoplanet Survey Satellite (TESS) and the Kepler Space Telescope, to capture these subtle fluctuations in luminosity by measuring the overall brightness of light coming from the star over the course of time. Since space-based telescopes are located above the Earth’s atmosphere, they are not affected by the climate and can be placed strategically according to the target star. This allows them to see fainter stars and measure the small brightness changes more accurately than ground-based telescopes.
Oscillation spectra are unique to each star, and they can be used to learn about the inner composition, stage of evolution, magnetic fields, and exoplanets of that host star.
Stellar Oscillations As Chronometers
Stellar oscillations serve as cosmic timekeepers, mirroring the star’s evolution. These oscillation frequencies are connected to the internal structure of stars and are predominantly influenced by their density and sound speed profiles. Their frequency shifts tell us a lot about the changes within the star’s core, similar to counting the rings of a tree to estimate its age.
For example, it is found that the p-mode frequency shifts in solar-type stars reduce as they grow older and their rotation periods increase.
Astronomers skillfully employ these frequency shifts to gauge a star’s age and refine theoretical models, deepening our understanding of stellar mechanisms and life cycles, even extending their reach into investigations of galaxy formation and the dynamics of stellar clusters.
Applications Of Asteroseismology
Stellar oscillations, or the movement of stars, not only affect the stars themselves, but also help us learn more about exoplanets and the life stages of a given star. If you think of a star and its planets as a playground seesaw, their gravitational pull on each other is somewhat similar to the gravity that keeps us on a seesaw—it causes a rocking or oscillating motion. This rocking motion helps us find and study exoplanets, even those we can’t see directly with telescopes.
The movements of stars provide tiny hints and clues about these hard-to-spot planets, which drives researchers to explore and learn even more about the hidden mysteries of outer space.
There are several uses of asteroseismology in the study of exoplanets, including:
- Determining the mass and radius of the star hosting an exoplanet. This is important because the mass and radius of the star determine the size of the habitable zone around the star, which is the region where liquid water could exist on the surface of an exoplanet.
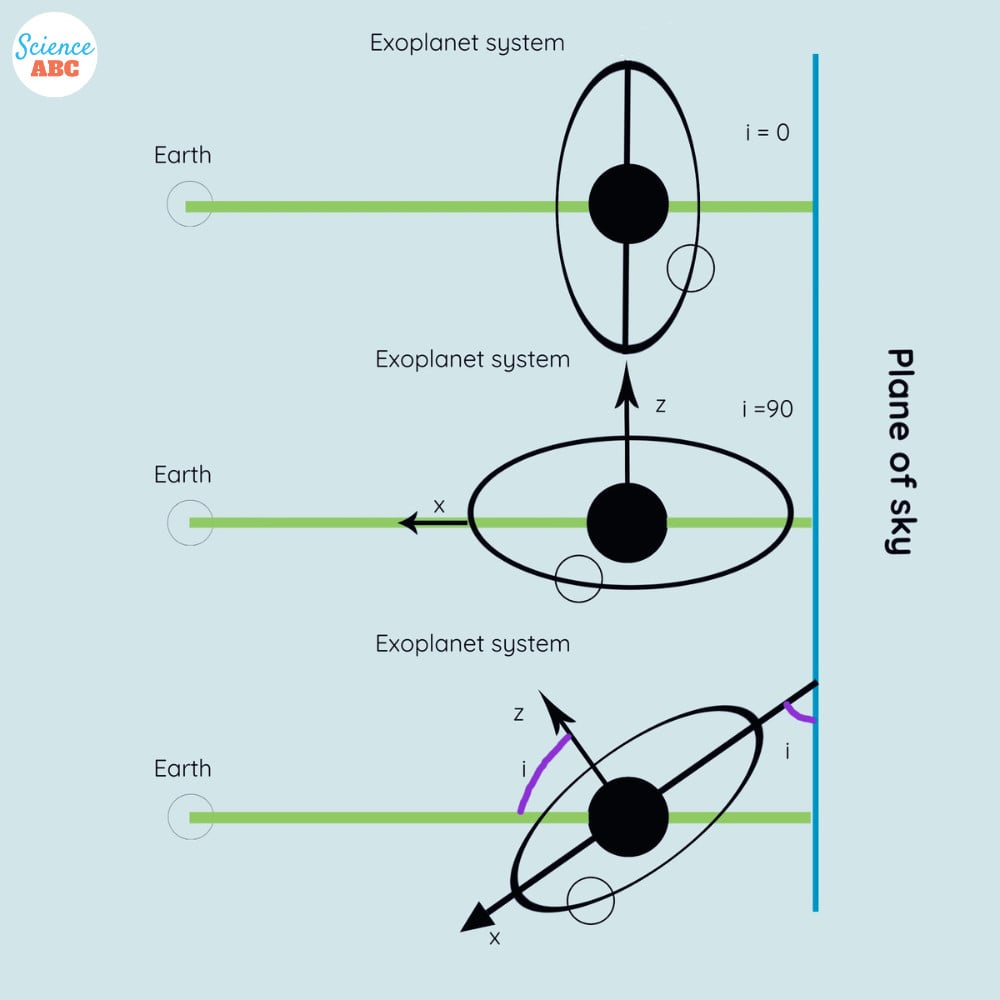
- Measuring the inclination of an exoplanet’s orbit. The inclination is the angle between the plane of the exoplanet’s orbit and the plane of the sky. This cannot be measured directly from transit observations, but it can be inferred from asteroseismology. If the inclination angle is zero degrees, the planet would pass directly in front of the star, and we would be able to see it transit the star. However, if the angle is 90 degrees, then the planet would never pass in front of the star, and observers on Earth would not be able to see it transit the star.
- Determining the eccentricity of the exoplanet’s orbit. The eccentricity is a measure of how elliptical an orbit is. Eccentric orbits are more elongated than circular orbits. The eccentricity of an exoplanet’s orbit can affect its climate and habitability.
In essence, asteroseismology grants us the ability to “listen” to the stars, not in the way we hear sounds on Earth, but in a manner that surpasses and extends our conventional senses. While the term “listening to the stars” is somewhat metaphorical, it reflects the idea that we can extract important physical data about stars by analyzing their oscillations through variations in stellar brightness. These oscillations open gateways beyond individual stars, broadening our view to encompass the cosmic horizon, and helping us in our search for potentially habitable exoplanets out there in the cosmos.
The interplay between stellar vibrations and the multifaceted arenas of astrophysics enriches our endless journey into the mysteries of our universe.
References (click to expand)
- Aerts, C., Christensen-Dalsgaard, J., & Kurtz, D. W. (2010). Asteroseismology. Astronomy and Astrophysics Library. Springer Netherlands.
- Chaplin, W. J., & Miglio, A. (2013, August 18). Asteroseismology of Solar-Type and Red-Giant Stars. Annual Review of Astronomy and Astrophysics. Annual Reviews.
- Symphony of stars: The science of stellar sound waves.