Neutron stars are some of the most bizarre and unique objects in the Universe. However, not much is known about these powerful remnants of dead stars.
In 1967, legendary astrophysicist Jocelyn Bell, a research student at the University of Cambridge at the time, was studying variations in radio signals in the data she obtained using a detector. The detector was constructed by her advisor, Antony Hewish, her doctoral advisor at the University of Cambridge, and a Nobel Prize-winning radio astronomer.
When she noticed sharp, rapid, and regular radio pulses coming from a source in the constellation Vulpecula, several astronomers thought the signals were of extra-terrestrial origin.
This radio source was called “little green men,” or LGM for short.
Later, they realized they had stumbled upon a particular type of neutron star. This class of neutron stars rotates extremely fast and are called pulsars. This was also the first ever observation of neutron stars (or pulsars).
Over the years, with increasingly better observational techniques, more kinds of neutron stars have been found. Presently, the number of neutron stars we have discovered stands at more than 2500.
So, what are these objects? And why are they called ‘neutron’ stars?
Recommended Video for you:
About Neutron Stars
In 1934, German astronomer Walter Baade and Swiss astronomer Fritz Zwicky first predicted the existence of neutron stars. However, there are some controversial reports that the Soviet physicist Lev Landau actually predicted it earlier in 1932, the same year neutron particles were discovered.
Baade and Zwicky proposed that when a dying star explodes as a supernova, the leftover iron core will collapse and become a neutron star. For this to happen, the mass of the star’s core should exceed the Chandrasekhar limit, which has a value of 1.4 times the mass of the Sun, or around 2.765*1030 kg.
Once the core collapses, the energy released by this collapse will cause the matter surrounding the core to be ejected. Once all of this surrounding matter gets blown away, the only remnant is the core, which goes on to become a neutron star.
As mentioned, more than 2500 neutron stars have been found. The one observed by Bell and Hewish is designated PSR B1919+21. Other famous ones include the Crab Pulsar, located in the Crab Nebula, and the Vela Pulsar, found in the Vela Supernova Remnant.
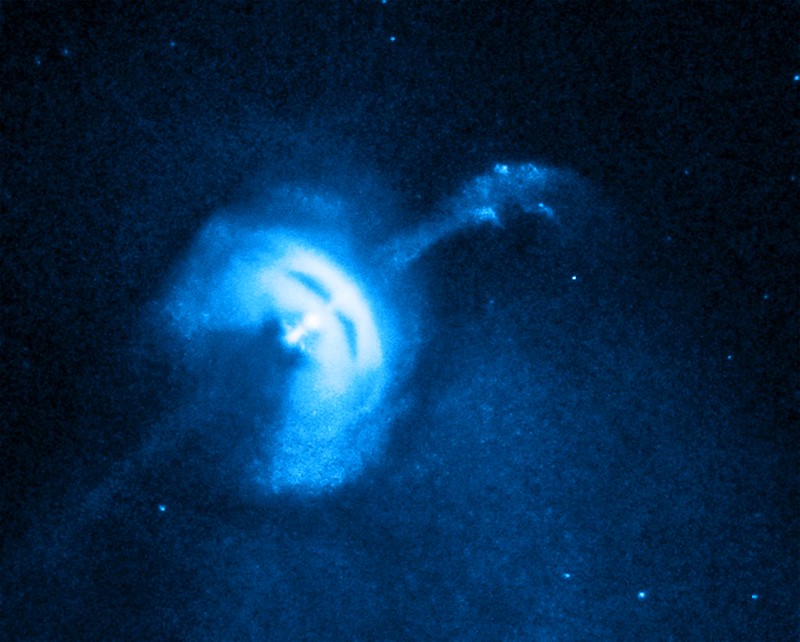
The name neutron star originates from the fact that they are composed almost entirely of neutrons. There are also trace amounts of an equal number of electrons and protons, giving the whole object a net neutral charge.
Neutron stars generally have a radius of only around 12 km. With masses exceeding 1.4 times that of the Sun, these are some of the densest objects in the Universe. A tablespoonful of neutron star material could weigh as much as Mount Everest.
Neutron stars also create some of the most powerful magnetic fields in the universe. The magnetic fields can be as much as one million billion (or one quadrillion) times stronger than that of Earth. In fact, in 2004, a neutron star, located around 50,000 light-years away, underwent an outburst. Its magnetic field was so strong that it even affected the Earth’s ionosphere, despite its colossal distance!
In such extreme environments, the matter inside neutron stars likely exhibit properties that are very different from what we usually see. This state of matter is called exotic quark matter. The material could display properties like superconductivity and superfluidity (superfluids are fluids that flow with zero viscosity).
To understand how neutron stars became like this, we need to know more about their formation, at a time when stars are just about to die.
Formation Of Neutron Stars
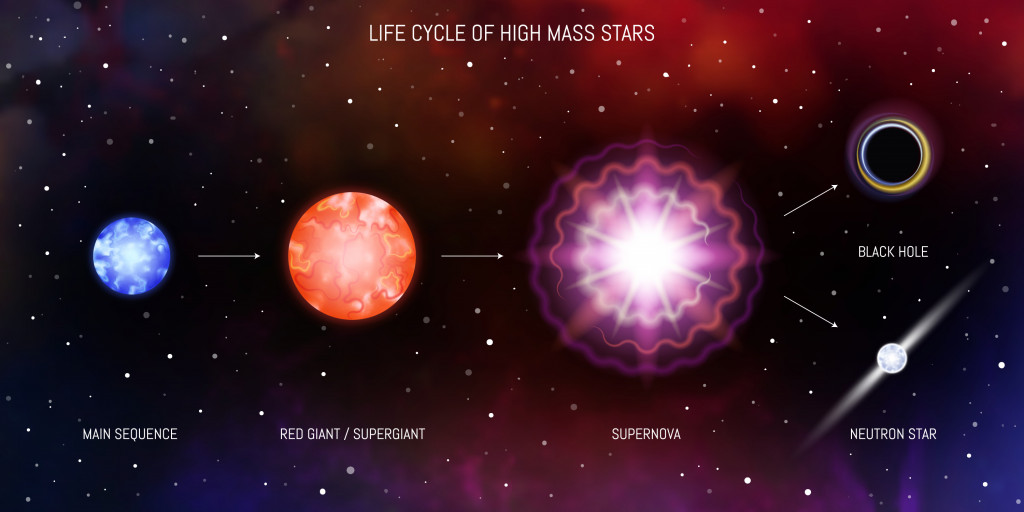
Stars undergo a number of stages during their lifetimes.
After a star is born, it enters a stage called the main sequence stage. This is the stage when the usual nuclear fusion process occurs in the star’s core. A majority of the stars we observe (including our Sun) are currently in this “main sequence”. In fact, stars live much of their lifetimes in their main sequence stage.
During its main sequence, a star attains stability because due to the equilibrium between two processes. One process is the gravitational force, which causes the star to collapse in on itself. The second process is thermal pressure, due to the nuclear fusion occurring in its core and moving outwards.
Since the inwards gravitational force is equal and opposite to the outwards thermal pressure, it creates an equilibrium and the main sequence star remains stable. This equilibrium is called hydrostatic equilibrium.
Towards the end of a star’s main sequence, its core finishes the fuel required to sustain the nuclear fusion process. Since no more thermal energy production takes place that opposes the gravitational force, the core collapses in on itself. With this collapse, the density and pressure increase, and the star expands into a supergiant.
Eventually, the high pressure causes the nuclei of iron and all other elements to start breaking down into smaller particles. A core massive enough (above the Chandrasekhar limit) will result in high values of density and pressure. In this case, a nuclear process occurs in which the protons and electrons in the core combine to form neutrons. This results in the emission of large amounts of energy, which we would see as a supernova.
Most of this energy is released in the form of kinetic energy. We see this in the expanding ejected matter, especially in the case of a type of particles called neutrinos and antineutrinos. These particles carry much of the energy and become irradiated in all directions, in nearly equal proportions. Meanwhile, electromagnetic radiation only takes up a small proportion.
After the supernova, the leftover core is rich in neutrons and becomes a neutron star.
As mentioned before, for a neutron star to be formed, the mass of the star’s core should exceed the Chandrasekhar limit. However, sometimes, the core of a star has so much mass (above another higher mass limit called Tolman–Oppenheimer–Volkoff limit) that gravity causes it to collapse to a point of infinite density. In that case, it becomes a black hole. The Tolman–Oppenheimer–Volkoff limit is a theoretical value that serves as the upper limit for the mass of a neutron star.
Thus, if the core of the star has a mass that is between the Chandrasekhar limit and the Tolman–Oppenheimer–Volkoff limit, it forms a neutron star. If the star’s core has a mass exceeding the Tolman–Oppenheimer–Volkoff limit, it forms a black hole.
Inside A Neutron Star
The interior of neutron stars consists of five major regions—the atmosphere, the envelope, the crust, and the inner and outer cores.
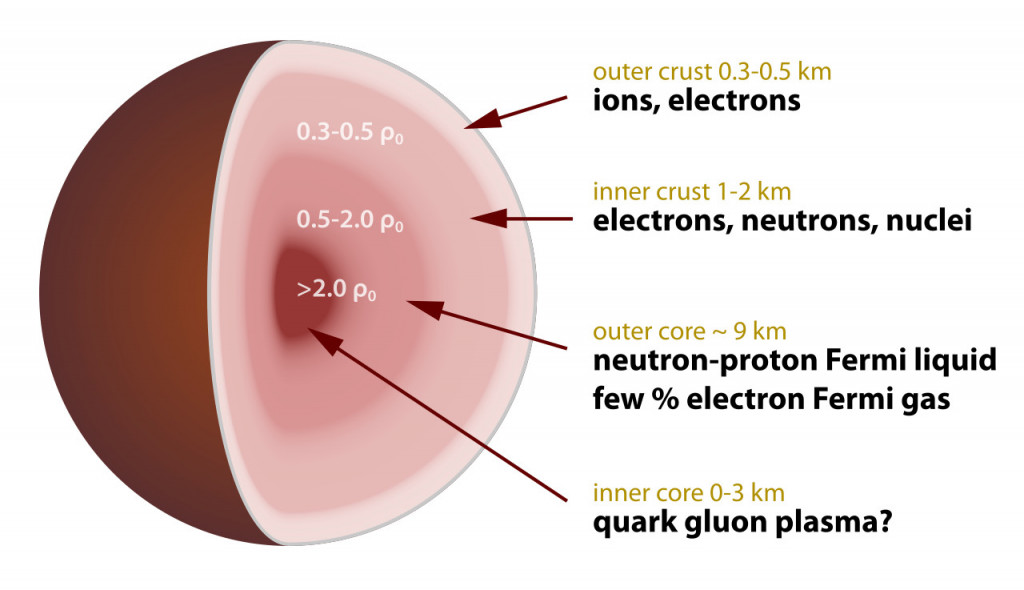
Theory predicts that the interior of neutron stars exhibits one of the strangest phenomena in the Universe. When we move towards the inside of a neutron star, its density also increases. This increase in density affects how the nuclei inside the neutron star are arranged.
At the crust-core boundary, the pressure is already so great that the nuclei almost touch each other. From there, the increasing densities cause the arrangement of atomic nuclei to change continuously in a bizarre fashion.
While the initial arrangement of nuclei has a 3-dimensional configuration (called meatballs), it progressively gets more flattened into 2-dimensional and then 1-dimensional layouts. These arrangements are given unique names like spaghetti, lasagna, ziti, and ravioli. It finally ends up as a uniform soup of nucleonic matter called “sauce”.
The core contains 99% of the neutron star’s mass, but has a very small diameter. The outer core consists of nucleons that show fluid-like properties (the neutrons, in particular, should show superfluidity). However, we do not have information about what happens in the inner core. The density is so high in the inner core that scientists have predicted the presence of exotic phenomena and strange states of matter.
Classification Of Neutron Stars And Pulsars
Neutron stars are classified either as isolated or as members of a binary system. Isolated neutron stars are neutron stars that freely float in space. These neutron stars emit some form of radiation, like visible, infrared, X-rays, or gamma rays.
Isolated neutron stars have several sub-classes. One such sub-class include compact central objects (COO), found at the center of supernova remnants. Another sub-class is dim isolated neutron stars (DINS), which are relatively older and emit X-rays. The most common sub-class, however, consists of pulsars.
Pulsars are rapidly rotating neutron stars that give a periodic pulse or signal. This pulse usually appears as radio emissions, but could be X-rays, optical, and gamma rays. While most pulsars spin roughly once per second, the fastest one ever observed spins around 650 times each second!
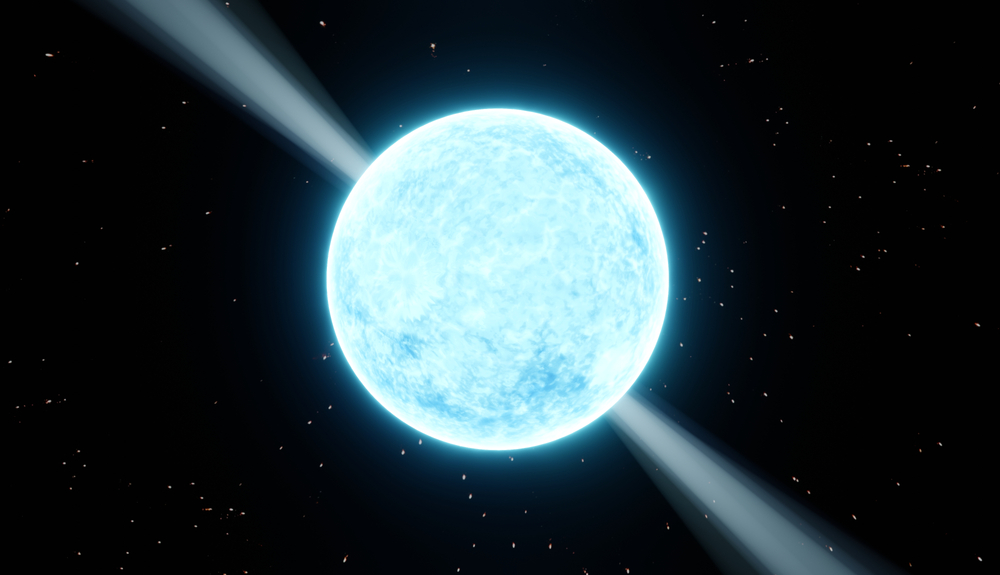
The reason for this high spin is because of a principle called conservation of angular momentum. Stars in their main sequence rotate about an axis, but when they collapse into a tiny object like a neutron star, the conservation of angular momentum causes it to spin even faster.
The pulses we see are emanations of radiation that emerge from a small area on the neutron star’s surface. As the neutron star spins, it sweeps this radiation across space. Whenever the beam is pointed in the direction of Earth, we see a pulse. It is similar to how a lighthouse functions—and has therefore often been called ‘The Lighthouse Model.’
Pulsars are some of the most common types of neutron stars we see. As mentioned, the first neutron star we observed, the one seen by Jocelyn Bell and Antony Hewish, was a pulsar. Currently, we have observed around 1600 pulsars.
Binary neutron stars are systems containing a neutron star that is gravitationally bound with an ordinary star. They are further divided based on the mass of the companion stars. If the companion is 2-3 times more massive than our Sun, it is a High-Mass X-ray binary (HMXB). If the companion is less massive than our Sun, it is a Low-Mass X-ray binary (LMXB).
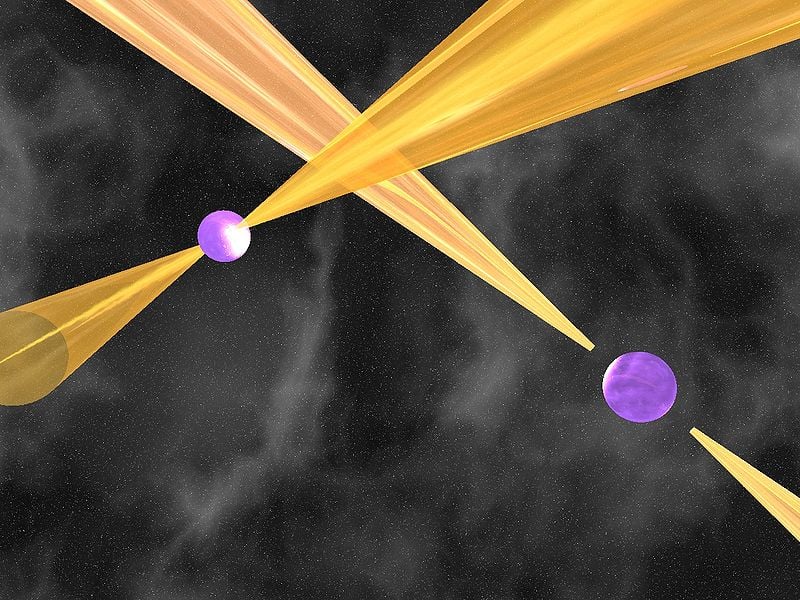
There has also been evidence of binary systems containing only neutron stars. When large binary stars both undergo supernovae, they might end up as two neutron stars orbiting each other. The system, PSR J0737-3039, consists of two pulsars orbiting each other.
Fate Of Neutron Stars
Neutron stars do not have any nuclear fusion process happening at their cores. Since there is no energy production, they eventually cool off and reduce the amount of radiation they emit. They also lose their spin and their magnetic field over time.
Binary neutron stars with a sufficiently close companion star will start accreting matter from the star itself. It will either result in the companion star becoming a white dwarf or a neutron star itself, but it could also lead to the destruction of the companion star.
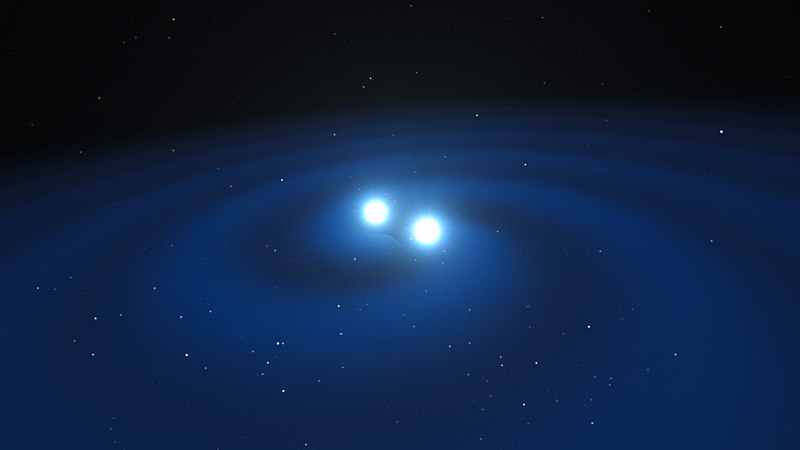
Sometimes, binary systems containing only neutron stars, will merge, resulting in a ‘kilonova.’ A kilonova is a type of explosion that lasts only for a short period. It generally occurs when compact objects like neutron stars and black holes merge. It is much less explosive than a supernova.
Mergers of neutron stars usually result in the formation of a new neutron star. However, if the merging neutron stars are massive enough, they will become black holes. Mergers of neutron stars have also produced powerful gravitational waves. The first observation of gravitational waves due to a neutron star merger happened on August 17, 2017.
Current And Future Prospects Of Neutron Stars
There is still a lot left to learn about neutron stars. We have some idea about their properties, which we have learned from pulsars and gravitational waves, primarily from their mergers. However, we still need to obtain observational evidence on properties like the maximum mass they could have, their radii, and their internal composition.
Neutron star mergers is one of the most popular topics in astronomy today. The gravitational waves emitted from these mergers contain information on their properties. The properties include information on their masses, their radii, the nature of their orbits, etc. These waves are observable over long distances. To observe these waves, detectors like LIGO, Virgo, and GEO600 are currently in operation.
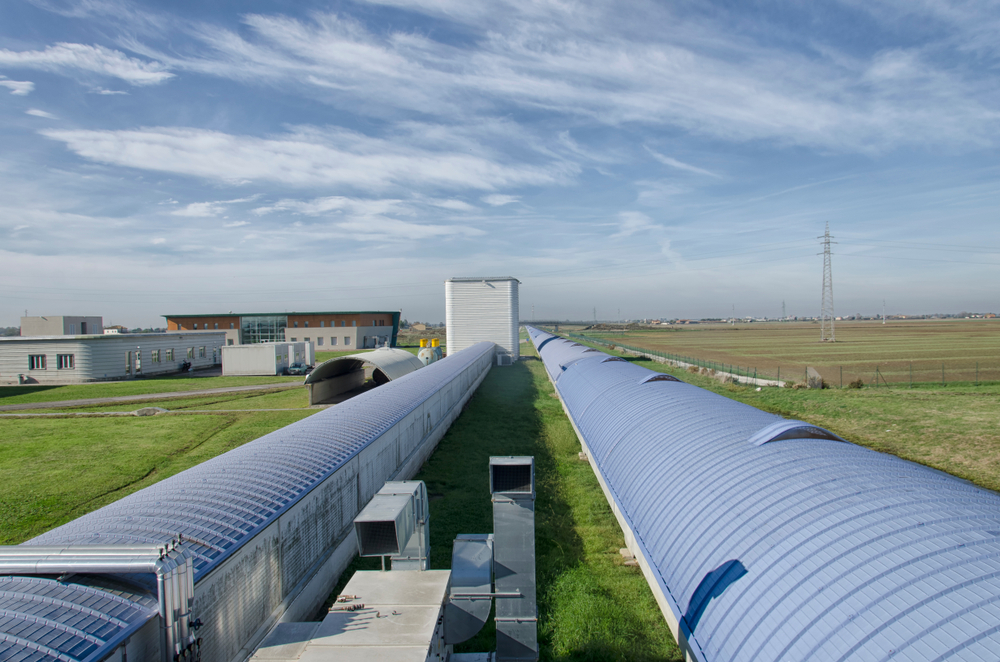
One study developed mathematical frameworks that could help us understand the properties of neutron stars in laboratories. The study includes using Helium superfluid, superconducting media, and ultra-cold gases. These types of studies help us understand the superconductive and superfluid nature of exotic matter found in neutron stars.
However, these studies also pointed out the difficulties of replicating neutron star environments in the laboratory. However, by combining the data we obtain from radio and gravitational observations of neutron stars, we could better understand what goes on inside these fascinating stars.
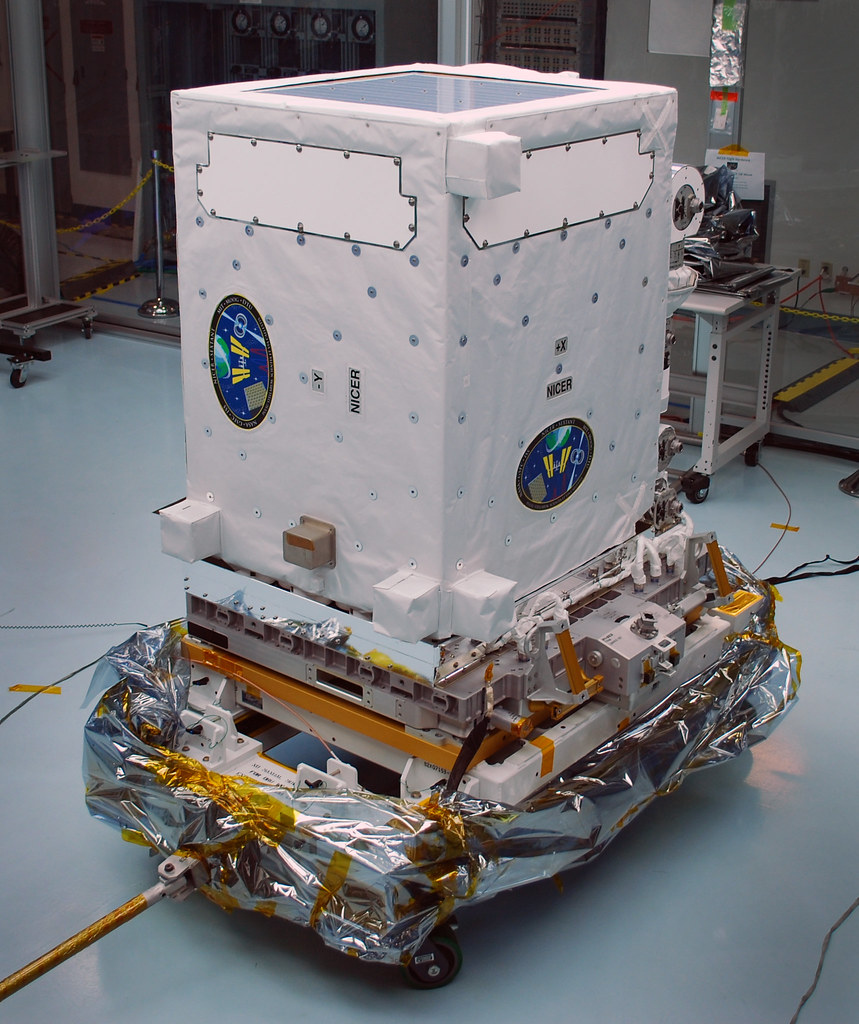
Recently, a program called the Neutron star Interior Composition ExploreR (NICER) was installed on the International Space Station in June 2017. The program aims to study the exotic matter present in neutron stars using its X-ray spectroscopy. It also aims to demonstrate the navigational capacities of spacecraft using pulsars.
Neutron stars can also provide a means of testing gravity. In particular, the binary pulsar PSR J1757–1854 is expected to provide the real-world laboratory for two tests on gravity.
One of the tests is on orbital deformation. It should tell us how much deviation should be present in elliptical orbits due to relativistic effects. The second test is about finding a precession, namely the Lense-Thirring precession, which has not been seen in binary neutron stars to date.
Neutron stars, alongside black holes, are some of the most extreme objects in the Universe. Studying such objects has helped us uncover new aspects of science, not only in astronomy but also in high-energy physics. It has helped us discover many things, including nuclear processes, new states of matter, and the eventual fate of the stars.
References (click to expand)
- 23.4 Pulsars and the Discovery of Neutron Stars – Astronomy. The University of Central Florida
- M Al-mamun. Neutron Star Structure from Electromagnetic and Gravitational .... The University of Tennessee system
- The Remarkable Properties of Neutron Stars | ChandraBlog. The Chandra X-ray Observatory
- AD Cameron. Current results and future prospects from PSR J1757-1854, a .... The SAO/NASA Astrophysics Data System
- Research — Binary Neutron Stars. The Center for Computational Relativity and Gravitation
- Pulsar | COSMOS - Centre for Astrophysics and Supercomputing. The Centre for Astrophysics and Supercomputing
- Lattimer, J. M., & Prakash, M. (2004, April 23). The Physics of Neutron Stars. Science. American Association for the Advancement of Science (AAAS).
- Neutron stars and pulsars - Hyperphysics. Georgia State University
- The Neutron Star Interior Composition Explorer Mission. The Goddard Space Flight Center